top of page
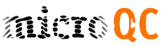
The three experimental groups USIEGEN, LUH and UOS focused on the technological development of large-gradient multilayer surface-electrode traps and the implementation of high-fidelity quantum gates and quantum algorithms with them. The two theory groups TCPA and HUJI supported the experimental ones in developing new or adapting existing quantum control strategies to the specific experimental conditions. A few representative examples of the activities follow.
LUH and USIEGEN developed a new geometry for a surface-electrode trap with embedded current-carrying wires (CCW) supporting the realization of strong gradients of up to 200 T/m. They also expanded their near-field simulation capability for microwave near-field conductors to implement a new scheme which brings together both the static-gradient and the near-field oscillating-gradient approaches. The new trap chip has been designed jointly by USIEGEN and LUH. Fabrication has been carried out in the patented multilayer fabrication process at LUH. In particular, LUH has been able to establish a lower bound of 13.6 (8.1) s on the coherence time of their field-independent qubit in the new setup without any shielding, refocusing pulses or dynamic decoupling.
LUH has systematically characterized and generalized pulse-shaped multi-qubit gates. They have developed a formalism to obtain optimized pulse shapes for a wide range of optimization targets. In the first period, LUH demonstrated their first two-qubit entangling gate with 98.2% fidelity and found motional-mode frequency changes to be the major contributor to the observed gate infidelity. They developed amplitude pulse shaping as a means of making the resulting gates more resilient to this type of noise by two orders of magnitude. To overcome timing limits of single-qubit gates, they used resilient pulse sequences developed by TCPA resulting in a final fidelity of 99.7%, which is no longer limited by motional mode instabilities. In the second period, further improvements in collaboration with TCPA have been made, using numerical optimization of the pulse shapes, which resulted in a speedup of the gate by a factor of 3, while maintaining its high fidelity.
A significant ingredient for the implementation of large-scale computing is the analysis of crosstalk between individually-addressed ions and the implementation of dedicated trap zones in a multi-ion processor. For microwave near-field quantum gates, LUH have implemented single-ion addressing using microwave micromotion sidebands. They achieved Rabi rates of 5.572(2) kHz and 0.01(5) kHz on two ions separated by just a few microns within the same potential well. This crosstalk can be further reduced through the application of optimized potentials. They have carried out randomized benchmarking on one qubit while measuring the effect on the other qubit, a technique pioneered by the USIEGEN group, and found an upper limit on crosstalk between the two ions of 6x10. For these developments, the collaboration with the TCPA group has been critical in the analysis of the relevant phases and parameter sensitivities of the implemented composite pulse sequences. Based on the single-ion addressing capability and the already demonstrated two-qubit gate, LUH have implemented cycle benchmarking of arbitrary two-qubit operations. This essentially implements a universal computation register for a shuttling-based ion trap quantum processor. The implementation of cycle benchmarking is also a critical step for the implementation of the gate operations in a computational context and will be key to a more thorough analysis of the two-qubit gate fidelity, as the analysis (compared to the tomographic characterization) is independent of the state preparation and measurement (SPAM) error.
In order to realize the qubit-qubit coupling based on the static magnetic field gradient, a novel approach based on a quasi-static permanent magnet has been developed and patented by USIEGEN. It is advantageous compared to both a permanent magnet as its field can be tuned and switched off, and to a system based on current-carrying electrodes as there are no running currents that create magnetic field noise. They demonstrated a gradient of 116 T/m while the field in the minimum is only 15 G. Applying adaptive frequency correction (resulting in a factor of 50 reduction in FWHM of frequency precision) together with using a different dynamical decoupling sequence led to an increase of the classical two-qubit CNOT fidelity from 0.79(4) to 0.97(6).
USIEGEN implemented a novel two-qubit phase gate, called AXY gate, generated by pulsed dynamical decoupling. The novel sequence protects two qubits from decoherence while generating a tunable two-qubit phase gate with high fidelity. The fidelity of this gate is robust against motional excitation, variations of motional frequencies, and amplitude variations in the driving field. The gate speed scales better than quadratically with the field gradient, an important feature in novel high-gradient traps. They have concluded, as implied by high-fidelity Ramsey results, that a Bell fidelity of 99% is reachable, even though it has not been independently measured yet.
USIEGEN reported the implementation of a simple quantum algorithm: the perceptron quantum gate in an ion-trap quantum computer. In this scheme, a perceptron’s target qubit changes its state depending on the interactions with several qubits. The target qubit displays a tunable sigmoid switching behavior becoming a universal approximator when nested with other perceptrons. They also used two successive perceptron quantum gates to implement a XNOR gate, where the perceptron qubit changes its state only when the parity of two input qubits is even. The applicability can be generalized to higher-dimensional gates as well as the reconstruction of arbitrary bounded continuous functions of the perceptron observables. This bodes well for future efforts to implement machine learning and deep learning algorithms on trapped-ion quantum computers, which have been demonstrated to yield significant speed-ups. USIEGEN have also demonstrated that quantum perceptrons can be used to implement tailor-made quantum gates, which would otherwise require a large single and two-qubit gate decomposition. Hence, this method could be used to reduce gate-count overhead in quantum algorithms.
The easiest way to raise quantum gate fidelities and gate speeds in a microwave driven ion trap is to increase the magnetic field gradient. Early experiments at UOS were carried out using a macroscopic ion trap setup including permanent magnets giving rise to a magnetic field gradient of 24 T/m. They have successfully developed a new generation of ion microchips with integrated current carrying wires. The microchips can obtain a magnetic field gradient of 145 T/m, contain X-junction ion trap arrays, and feature low resistance integrated current carrying wires. They have successfully trapped ions using these ion chips demonstrating lifetimes of 7-16 hours. The expected two-qubit gate fidelity depends on the magnitude of the magnetic field gradient, and the expectation is to demonstrate fidelity of over 99.9% with this magnetic field gradient (previously 98.5% with 24 T/m).
At UOS, making use of new ion microchips with current carrying wires along with quantum control techniques developed and new gate mechanisms, MicroQC has been successful in developing all key ingredients for high fidelity two-qubit gates using static magnetic field gradients. New gate mechanisms have the potential to make quantum gates more robust, mitigate the requirement of ground state cooling and to enable larger values of entanglement fidelities. UOS have developed a new gate mechanism featuring a number of significant advantages. It does not require ground state cooling, in contrast to most other quantum gates, and it can achieve really high gate fidelities only making use of Doppler cooling. This removes a significant overhead for the practical operation of quantum computers. The gate is also extremely robust enabling extremely high gate fidelities. Making use of a magnetic field gradient of 150 T/m along with the implementation of a phase flip, it is possible to execute gates with a duration of 345 µs with errors below 10.
UOS have worked on developing a full toolbox of ion transport operation on our X-junction ion trap array. Such ion transport operations are required for the implementation of quantum algorithms within a transport-based quantum processor. This approach is alternative and superior to the photonic based approaches, as it makes use of electric field links between quantum computing modules instead of photonic interconnects. In this approach the electrodes on the edge of the microchip are shaped so that one can simply transport an ion from one microchip to an adjacent microchip. They have developed a full library of ion transport operations referred to as shuttling primitives, covering all shuttling operations. These primitives are added to give rise to an arbitrary shuttling operation. Each shuttling primitive is developed via a simulation engine which creates voltages sequences making use of electric field simulations and relevant constraints in order to arrive at relevant voltage wave form capable of implementing each shuttling primitive. Following the successful construction of this two-module quantum computer prototype, UOS achieved the demonstration of ion transport between two quantum computing modules at a transfer rate of 2424 s with ion transfer fidelity of 99.999993%, both of which are currently the state of the art.
Finally, UOS conducted comprehensive research on the Roadmap of microwave quantum computation to high-technology readiness level. An efficient ion routing algorithm has been created along with an appropriate error model, which can be used to estimate the achievable circuit depth and quantum volume. Making use of this analysis, they aim the roadmap for a large scale quantum computer to cater for a qubit number of 106 along with a base error rate of 10 as a useful starting point as resource specification for a universal transport-based quantum computer.
-4
-4
-1
-1
bottom of page